The Process of Translation in Protein Synthesis
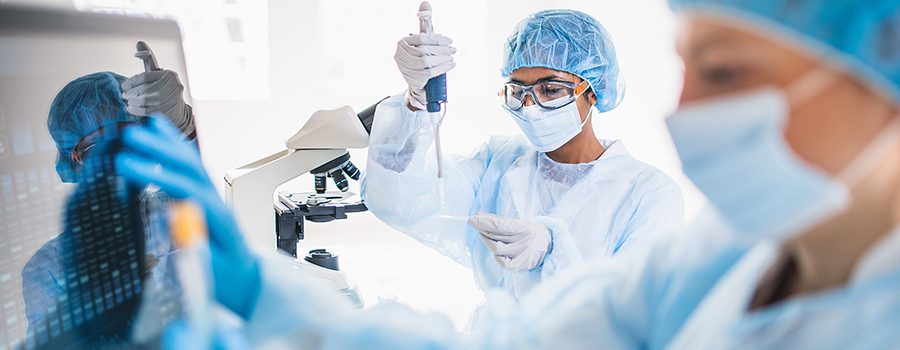
The process of translation in protein synthesis is significant for the intricate functioning of cells and serves as the mechanism through which the genetic code (carried by messenger RNA, mRNA) is effectively converted into functional proteins. In this process, specialized cellular machines called ribosomes meticulously read the genetic code embedded in the mRNA and use transfer RNA (tRNA) molecules to bring specific amino acids, assembling them into a polypeptide chain. The resulting protein’s structure and function are important for the proper functioning of the cells, making translation an indispensable contributor to various biological processes essential for life.
Overview of protein synthesis
Protein synthesis is a highly intricate and regulated cellular process that is central to biology and genetics. It involves a series of complex steps related to molecular biology. The process begins within the cell’s nucleus, where DNA gets transcribed into messenger RNA. This newly formed mRNA then undergoes translation. During this translation process, ribosomes read the genetic code encoded in the mRNA, which is organized into triplet sequences called codons. Then, tRNA molecules serve as couriers and deliver the right amino acids to the ribosome, assembling them in a precise sequence to form a polypeptide chain. This chain is the building block of proteins.
Transcription
Transcription is a fundamental biological process responsible for converting DNA into RNA. This pivotal step in gene expression allows the genetic information stored within DNA to be utilized for various essential cellular functions.
During transcription, a specific segment of DNA acts as a template and helps create a complementary RNA molecule. This mRNA carries the genetic instructions and transports them from the DNA to the ribosomes, where protein synthesis occurs. Other types of RNA, such as tRNA and rRNA, are also transcribed from DNA and are vital in the translation process.
At the core of transcription lies the enzyme RNA polymerase. This enzyme recognizes and binds to a specific DNA sequence called the promoter region. Once bound, it unravels the DNA’s double helix structure and begins assembling an RNA strand that complements the DNA template. RNA polymerase catalyzes the formation of phosphodiester bonds between RNA nucleotides, which creates a growing RNA chain.
Translation
The process of translation turns genetic information contained in RNA into functional proteins. It involves three key stages, namely initiation, elongation and termination. In the initiation phase, a small ribosomal subunit attaches to the mRNA molecule, guided by specialized initiation factors. During elongation, the ribosome moves along the mRNA to read the codons (genetic code) and add the corresponding amino acids to form a growing protein chain. This process relies on tRNA molecules that carry the amino acids. Finally, termination marks the end of translation when a stop codon is encountered, releasing the newly synthesized protein.
Initiation
The initiation stage of the translation process is the starting point for protein synthesis and hinges on forming a complex molecular structure. The small ribosomal subunit is at its core, which is significant in this process.
During initiation, the small ribosomal subunit initially recognizes a specific region on the mRNA molecule. In prokaryotes, this region is known as the 5' untranslated region (UTR), while in eukaryotes it’s the 5' cap of the mRNA. This recognition is essential, as it ensures the accurate placement of the ribosome on the mRNA.
In prokaryotes, the small ribosomal subunit carries an initiator tRNA molecule loaded with methionine and binds to the Shine-Dalgarno sequence on the mRNA. In eukaryotes, the small ribosomal subunit attaches to the mRNA’s 5' cap, guided by specific initiation factors.
Once the small ribosomal subunit is correctly positioned, the large ribosomal subunit joins it to form a functional ribosome. This assembly helps complete the initiation complex and paves the way for the onset of protein synthesis.
Elongation
Elongation is a second stage in the translation process, where the ribosome actively constructs the growing polypeptide chain while traversing the mRNA strand. This step involves various intricate processes, including introducing amino acids and forming peptide bonds.
As the ribosome moves along the mRNA in a 5' to 3' direction, it reads the mRNA’s codons. Each codon represents a specific amino acid, which is transported to the ribosome by specialized molecules called transfer RNA. These tRNAs possess anticodons that precisely match the mRNA codons, ensuring the correct amino acid is added to the growing polypeptide chain.
Elongation factors play a vital role in this phase. They help correctly position incoming aminoacyl-tRNA and assist in the creation of peptide bonds between the amino acids. The ribosome’s peptidyl transferase activity catalyzes the formation of these bonds, effectively connecting the newly added amino acid to the expanding chain. The ribosome then moves along the mRNA to the next codon, repeating this stepwise elongation until a stop codon is encountered. This stop codon serves as a signal for the termination of translation.
Termination
Termination is the third and final translation stage, responsible for the precise conclusion of protein synthesis. It is a highly orchestrated process guided by specific stop codons and the participation of release factors.
During translation, the ribosome progresses along the mRNA strand and ultimately encounters a stop codon (UAA, UAG or UGA) within the mRNA’s coding region. These stop codons do not code for any amino acids. Instead, they act as signals to stop the ongoing protein synthesis.
Release factors serve their role in the termination process. When a stop codon is identified, a release factor binds to the ribosome’s A site. This binding triggers the release of the fully formed polypeptide chain from the ribosome. At the same time, it disassembles the ribosome complex to release the ribosomal subunits and the mRNA.
Termination ensures the precise and timely conclusion of protein synthesis. The inherent specificity of stop codons, including their accurate recognition by release factors, guarantees that translation stops precisely where it should.
Post-Translation Modifications
Post-translation modifications play an important role in shaping the structure and function of proteins. These modifications cover a wide range of chemical changes that occur after a protein has been synthesized while adding complexity and diversity to the proteome, which encompasses all the proteins expressed in a cell or organism. PTMs exert a profound influence over how proteins behave and what roles they play in biology.
PTMs can involve adding or removing various chemical groups, such as phosphorylation, glycosylation, acetylation, methylation and ubiquitination, among others. These modifications can alter a protein’s conformation, stability, subcellular localization or interactions with other molecules. They can also regulate enzymatic activity, signal transduction and protein degradation.
The significance of PTMs becomes apparent considering their involvement in countless cellular processes, spanning gene expression, cellular communication, immune responses and metabolism. They enable proteins to perform precise functions exactly when and where needed, contributing significantly to the incredible adaptability of biological systems.
Analyzing translation efficiency
The analysis of translation efficiency involves experimental techniques like ribosome profiling and fluorescence-based assays to determine translation efficiency. These methods offer insights into translation rates and dynamics and shed light on gene expression mechanisms.
Several factors influence translation efficiency, including codon usage bias, RNA secondary structure and cellular conditions. As a result, it is important to understand translation efficiency to interpret gene expression intricacies and optimize protein production. Moreover, it’s applicable to various fields, spanning biotechnology, therapeutics and synthetic biology.
Experimental Techniques
Several experimental methods offer valuable insights into translation rates and efficiency. These experimental techniques serve as invaluable tools for delving into the complexities of translation efficiency. Here are five of these techniques:
Ribosome Profiling
Ribosome profiling (known as Ribo-seq) is a potent technique offering in-depth insights into the translation efficiency at the transcript level. This method involves deeply sequencing ribosome-protected mRNA fragments, providing a comprehensive view of actively translating ribosomes and revealing their precise positions along mRNA molecules.
By comparing the quantity of these ribosome-protected fragments to the total mRNA levels, researchers can determine how effectively a specific mRNA is being translated. Ribo-seq is important to investigate the dynamics of gene expression, pinpoint translation initiation sites and uncover the regulatory mechanisms influencing the pace of protein production.
Fluorescence-Based Translation Assays
Fluorescence-based translation assays are cutting-edge tools that enable researchers to monitor real-time translation processes. These assays frequently use proteins or ribosomes tagged with fluorescent labels by incorporating techniques such as fluorescence resonance energy transfer (FRET) or fluorescent proteins.
They help track translation events by observing changes in fluorescence intensity. As a result, they not only allow the measurement of protein synthesis speed but also detect translational pauses and investigate the factors that influence translation efficiency. These assays have transformed our ability to examine the dynamics of translation and provide critical insights into how cells regulate protein production.
Factors Affecting Translation Efficiency
Translation efficiency is intricately regulated by several factors, two of which are codon usage bias and RNA secondary structure. Codon usage bias requires preferring certain synonymous codons, which directly impacts translation speed. The specific codons chosen can either accelerate or impede this process by affecting the availability of tRNA molecules. On the other hand, RNA secondary structure (with elements like stem-loops or hairpins) can act as obstacles during translation. Such structures hinder ribosome binding or slow down the translation process by creating roadblocks along the mRNA molecule.
Codon Usage Bias
Codon usage bias is a genetic phenomenon where specific synonymous codons (those that code for the same amino acid) are favored over others in the protein-coding regions of genes. This bias can vary across species, genes and even within different sections of the same gene.
The choice of synonymous codons can impact translation speed and efficiency. Some tRNAs are more abundant in cells than others. Codons that correspond to these abundant tRNAs are typically used more frequently, facilitating faster translation due to readily available tRNAs. This phenomenon is known as optimal codon usage.
Understanding codon usage bias is vital as it provides insights into the factors that affect the efficiency and accuracy of protein synthesis. Factors such as natural selection, mutation tendencies and the kinetics of translation all influence these codon preferences. Researchers can study these preferences to improve the expression of foreign genes, design efficient synthetic genes and gain a deeper understanding of the intricate processes involved in translation across different organisms.
RNA Secondary Structure
RNA secondary structure encompasses the way single-stranded RNA molecules, including mRNA , fold and interact with themselves. These structural patterns play a critical role in controlling gene expression and can significantly impact various stages of protein synthesis.
In the initiation phase of protein synthesis, stable RNA secondary structures like stem loops or hairpins found in the 5' untranslated region of mRNA can hinder the binding of ribosomes. This obstruction affects the efficiency of ribosomes attaching the mRNA, potentially causing a delay in the onset of protein production.
Throughout the elongation stage of translation, RNA secondary structures within the coding region can also present challenges. Ribosomes might slow down or even pause when they encounter these stable structural elements, impeding their movement along the mRNA.
Moreover, RNA secondary structure acts as a vital regulatory component in gene expression. It can control how easily ribosomes and other translation-related molecules access specific mRNA sequences, either enhancing or inhibiting translation. This regulatory mechanism allows cells to fine-tune protein production in response to changing conditions or developmental stages, ensuring precise and controlled gene expression.
Ribosome Binding Sites
The accessibility of the ribosome binding site (RBS) on mRNA is a vital factor determining how efficiently translation occurs. The RBS is the specific region where ribosomes initially attach to mRNA, marking the starting point of translation. Several factors come into play when it comes to influencing the accessibility of the RBS, and these factors have a direct impact on the speed at which translation initiation happens.
One significant factor in this process is the presence of RNA-binding proteins. These proteins can attach to specific segments of mRNA, either aiding or obstructing ribosomes’ access to the RBS. Some regulatory proteins act as facilitators, ensuring that translation initiation proceeds smoothly, while others serve as repressors, causing delays or even inhibiting the initiation process.
Another important aspect is the influence of RNA secondary structures. These structures, like stem-loops or hairpins found in mRNA, can physically block the RBS, making it difficult for ribosomes to bind. However, RNA helicases, specialized enzymes, can unwind these structures, making the RBS more accessible.
Understanding and being able to manipulate RBS accessibility is of utmost importance, especially in optimizing translation efficiency in fields such as biotechnology and therapeutics. By adjusting these factors, researchers can finely control gene expression and improve the production of specific proteins as needed.
Future perspectives and applications
The future of translation efficiency research holds immense promise, with far-reaching applications across multiple domains. An in-depth understanding of these fundamental processes will bring about innovative biotechnology, therapeutics and synthetic biology developments. Here are five future applications:
Biotechnology Advancements
A thorough research into translation efficiency can revolutionize biotechnology by enhancing the design of more efficient systems for expressing recombinant proteins. This optimization will result in increased yields of therapeutic proteins, enzymes and biofuels. These advancements can potentially reduce production costs, enhance the availability of crucial proteins and support the development of sustainable energy sources.
Gene Editing and CRISPR Technology
A deeper understanding of translation efficiency can significantly benefit gene editing and CRISPR technology. It allows for fine-tuning guide RNA design and delivery, which leads to improved precision and effectiveness in CRISPR-based gene editing. This, in turn, raises the success rate of genetic modifications, opening up new possibilities in gene therapies and genetic research.
RNA-Based Therapeutics
Insights into translation efficiency are vital in advancing RNA-based therapeutics, including mRNA vaccines and gene therapies. By optimizing the delivery and expression of therapeutic RNA molecules, researchers can enhance the effectiveness and safety of these treatments. This holds immense promise for addressing various diseases and advancing personalized medicine.
Synthetic Biology
Synthetic biology applications stand to benefit significantly from the fine-tuning of translation efficiency. This knowledge helps scientists precisely engineer intricate genetic circuits and artificial biological systems. From creating synthetic organisms to developing biosensors, these advancements enable the design of innovative solutions to a wide range of biotechnological challenges.
Drug Development
The targeted modulation of translation efficiency holds great potential in drug development. Researchers can utilize small molecules or therapeutic interventions to influence translation rates, allowing for the design of more effective drugs. This approach offers opportunities to develop novel treatments and improve the efficacy of existing medications, ultimately advancing healthcare and pharmaceutical science.
Conclusion
To sum up, translation efficiency is important in the intricate gene expression and protein synthesis processes. Many mechanisms govern translation efficiency, including factors like codon usage bias and RNA secondary structure. Moreover, the essential experimental techniques are also leveraged in this field.
This knowledge holds immense potential, with applications extending to biotechnology, therapeutic development and synthetic biology. As a key factor in shaping gene expression, the process of translation in protein synthesis drives significant advancements in science and innovation.
You can revolutionize your laboratory operations with dedicated equipment and expertise from Avantor. Learn more about the role of RNA in driving protein synthesis and propel your scientific pursuits forward.